Sidney Perkowitz delves into the cutting-edge work being done to make brain–computer interfaces safer, more durable and widely available
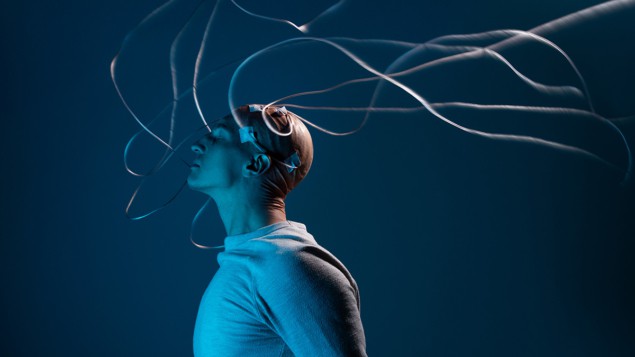
The human brain is an astounding and complex piece of machinery. With more than 80 billion neurons in the human cerebral cortex, each with a thousand synapses, our brains process some 100 megabits of information per second. Imagine then, attempting to measure, extract and interpret all the signals in our brain in real time, at the speed of thought. Tapping into the brain might once have been solely in the realms of science fiction – from X-Men to The Matrix – but today, it’s actually possible to link your brain to a computer and control a robotic arm, say, or translate your thoughts into text.
A brain–computer interface (BCI) functions as a bridge between your brain and an external device, typically a computer. BCIs collect, analyse and translate electrical signals from your brain into commands that can be understood and executed by a computer. They can also apply external signals to modulate the brain. Thanks to a combination of neuroscience, biomedicine, physics and technology, BCIs can change the lives of people with serious medical conditions. They also have applications across robotics, neuroscience, technology, gaming and computing.
Over the last 25 years, BCIs have allowed paralysed people to operate computers by thought alone. They have restored speech after it has been lost due to a stroke; have allowed those with missing or paralysed limbs to function again or helped them to operate robotic arms and hands. BCIs have diagnosed epilepsy and other neurological conditions, and mitigated them for tens of thousands of people. They’ve even shown promise for restoring sight to the blind.
But most of these examples require brain surgery, in which electrodes are placed on or in the surface layer of the brain (the cortex) and potentially even more deeply, which is risky as it could induce haemorrhages or infections. Another problem is that researchers currently don’t have a clear idea of the impact and potential damage the implanted electrodes could inflict on brain tissue, while also not knowing how long they may last. All this means that electrical implants in their current state cannot safely and reliably help the millions who would benefit from them. In fact, human implantations are carried out only when all other treatment fails, or on an experimental basis – for some 50 individuals worldwide with severe limitations such as paralysis – where the chance to improve a poor quality of life outweighs the dangers.
Fortunately, the solutions to some of these issues may lie in physics principles and methods, which could make these devices safer, more durable and more widely available. Physics could also be used to improve BCI implantation methods and materials. More crucial though, is the need to eliminate or minimize brain surgery by providing ways to interact with the brain via light, magnetic fields or ultrasound. Non-invasive, wireless and portable or wearable BCIs could enhance brain research and medical treatment, and be used in daily life as well.
Tap in with a thought
From antiquity through to the 19th century, physicians and experimentalists, often unwittingly, carried out various rudimentary experiments in which they tried to modify the brain’s electrical activity for medical treatment. In 1924 these efforts became rigorous when German psychiatrist Hans Berger recorded electrical brain activity by using electrodes placed on a patient’s skull, thereby inventing the technique of electroencephalography (EEG). In the 1970s physicist and computer scientist Jacques Vidal demonstrated thought control of an external device, as human subjects fitted with EEG contacts mentally moved a cursor displayed on a computer screen.
EEG remains a valuable non-invasive tool to diagnose conditions such as epilepsy, allowing us to determine the cause and type of seizures a patient might be suffering from, as well as to investigate other conditions such as dementia, brain tumours and concussions. But an EEG samples large groups of neurons, and the signal-to-noise ratio is poor, making it difficult to correlate the signals with specific brain activities.
Implanted electrodes, on the other hand, directly sample selected neurons. This was experimentally demonstrated in 1998, when Atlanta-based neurologist Philip Kennedy placed custom-designed electrodes into the brain of a patient dubbed “JR”, who had been left “locked-in” by a stroke (IEEE Trans. Rehabil. Eng. 8 198). The unlucky patient was in possession of his full cognitive abilities, but unable to move or speak. Eventually, JR learned to communicate by mentally controlling a computer cursor to spell out words.

Now many researchers and clinicians use an implanted electrode array, known as the “Utah Array” from Blackrock Neurotech. This tailored silicon product is an array of 100 p-type silicon electrodes (in a 10 × 10 configuration), spaced 400 µm apart on a 4 × 4 mm insulating substrate – roughly the size of a peppercorn. The electrodes, 0.5 to 1.5 mm long, are tipped with platinum or iridium oxide. Some 30 people across the world, suffering with different symptoms of paralysis, have been fitted with these devices. For example, in 2015 four arrays were implanted in Nathan Copeland, who was paralysed from the chest down after a car accident in 2004. The implants allow him to control a computer, play video games and control a robotic arm, with his thoughts. At the time of writing, Copeland is the longest-running patient with such an implant, but the truly long-term implications of this invasive technology are not fully understood.
Reducing invasiveness
The trouble with an electrode or any other artificial implant in the brain is that it can trigger an immune response, which inflames and scars nearby tissue. This is aggravated by the mechanical mismatch between a rigid electrode and the brain’s soft tissue, which in turn can also degrade the electrode’s performance.
Finding durable, biocompatible materials with suitable electrical properties for electrodes and substrates is a challenge for physics and materials science
But finding durable, biocompatible materials with suitable electrical properties for electrodes and substrates is a challenge for physics and materials science. Promising candidates include soft and flexible conducting polymers, as well as extremely thin electrical conductors such as carbon nanotubes and silicon nanowires (for another approach, see box below).
Researchers are also working to reduce surgical risks, by adapting existing medical technologies. Stents – tiny hollow cylinders – are commonly used to hold open various types of vessels in the body. In one common use, they keep coronary arteries open and are considered minimally invasive. Neurotech company Synchron has developed “stentrodes” (stent-electrode recording array). They are electrodes mounted on a stent that is permanently implanted into a blood vessel in the brain. They can detect brain signals and wirelessly send them to a computer. In human trials, stentrodes have allowed paralysed subjects to operate computers (J. NeuroIntervent. Surg. 13 102).
Using a different approach, the US firm Neuralink announced in 2019 that it had developed a BCI that would be implanted flush with the skull by a surgical robot, which would also place 1024 or more flexible electrodes into the brain (J. Med. Internet Res. 21 e16194). Neuralink, which was co-founded by Elon Musk, has not published more details since, but after its recent approval from the US Food and Drug Administration (FDA) for human trials, more information may be forthcoming. In whatever forms, electrode-based BCIs will continue to be important because of their high spatial resolution and fast response, but non-invasive approaches are also rapidly developing.
Fibres, nanoparticles and the brain

Polina Anikeeva is an interdisciplinary scientist at the Massachusetts Institute of Technology (MIT), who works across materials science, brain research, and electronics. Following a BSc in biophysics in Russia, her PhD at MIT focused on organic LEDs and nanoparticles. As a physicist trained in fundamental concepts, Anikeeva realized that she could choose to work on “whatever interested” her that also allowed her to make a difference.
That turned out to be neuroscience working at Stanford University with Karl Deisseroth, who developed optogenetics, a breakthrough technique to control the activity of neurons with light. There for the first time she held a brain in her hands. It wasn’t firm, but soft “like pudding”. This generated an “aha” insight as she realized that physical probes of the brain should match its material properties for minimal invasiveness and maximum stability. Her answer was to fabricate flexible multifunction fibres, tens of microns in diameter, that could stimulate neurons either by using light or by delivering drugs to the patient and electrically recording the responses. After wide use to examine brain function in rodents, Anikeeva and co-workers now report (bioRxiv:2022.10.09.511302) the first use of her fibres to study brain function in non-human primates. This is an initial step toward human trials.
Anikeeva also draws on her materials background to study nanoparticles as sources of brain stimulation. Her results show that magnetic nanoparticles activated by an external field can affect deeper parts of the brain than is possible with “transcranial magnetic stimulation”, where a varying magnetic field applied to the skull induces currents in the neurons below.
Along with her research, Anikeeva and several colleagues have called for developing attitudes toward responsible neurotech within the science and engineering community. Combined with appropriate regulation, she believes this would help individuals and society navigate the ethical questions raised by neurotech and its medical uses such as BCIs.
Photons probe the brain
In the electromagnetic spectrum, near-infrared (NIR) light, which runs from 700 to 1400 nm, can traverse the skull and penetrate the brain centimetres deep, without doing harm, so long as the power density is held to milliwatts per square centimetre. A non-invasive NIR method dubbed “photobiomodulation” has shown that it can stimulate the brain. For instance, in a clinical trial in 2021, patients with dementia were repeatedly exposed to LEDs emitting light at 1060–1080 nm. This group showed notable improvements in cognitive function and subjective mood compared to a control group (Aging Dis. 12 954). It is thought that the light enhances cellular function or reduces inflammation, but more research is needed to establish the exact mechanism.
A second non-invasive method, known as “functional near-infrared spectroscopy” (fNIRS), uses NIR light to measure variations in the light absorbed by haemoglobin in the blood circulating in the brain. The technique can map brain activity because deoxygenated haemoglobin absorbs NIR light differently from the oxygenated form, HbO2. Active neurons need an increased flow of HbO2-enriched blood, making it possible to detect brain function. Two wavelengths are applied to the skull, and a measurement of their different attenuations at specific sites can show which areas are active. fNIRS has been used in the clinic, with US neurotech company Kernel developing a wearable headset version. It covers the skull with 52 modules, each with laser sources emitting at 690 nm and 850 nm and a detector (J. Biomed. Opt. 27 074710). In 2021 the FDA approved the device to test the brain’s response to a psychedelic drug.

Although it takes seconds for the oxygenated blood flow to develop – making fNIRS too slow to control an external device – it does deliver a higher spatial resolution and better signal-to-noise than EEG, meaning it can pinpoint brain activity more accurately. An fNIRS headset could measure brain activity even in a freely moving subject, making it possible to map the brain and diagnose neural conditions under varying conditions.
Faster responses can be obtained with another method – known as “event-related optical signal” (EROS) – that uses infrared light to measure changes in the optical properties of cortical brain tissue. The interaction of light with neural tissue changes when neurons are active because that increases the optical scattering, lengthening the paths of photons traversing the brain and delaying their arrival at a detector.
In early experiments on human subjects, NIR light applied through optical fibres penetrated the skull and was detected a short distance away, delayed by 0.1 s or less after neurons had been excited. Further work has been limited because these measurements are technically demanding, but recent results suggest that EROS combined with fNIRS could form the basis for non-invasive BCIs with good spatial and temporal resolution.
The magnetic brain
Yet another established non-invasive method to trace the brain’s neural activity is “functional magnetic resonance imaging” (fMRI). Standard MRI detects the behaviour of protons in water and fat in the body, within a strong magnetic field, to image bodily structures. fMRI instead detects signals from blood flow in the brain that, as mentioned, depend on the oxygenation level of haemoglobin. Like fNIRS, this allows fMRI to label regions of neural activity but at a spatial resolution of 1 mm rather than 1 cm. The time lag of seconds allows mapping nearly in real time, but it is still too slow for brain control of external devices. fMRI also requires a large, expensive installation with a superconducting magnet.
Faster response times come with non-invasive “magnetoencephalography” (MEG), which tracks neural activity by detecting the femtotesla (10–15 tesla) magnetic fields that are generated as ionic currents flow among active neurons. These fields are measured by sensitive superconducting quantum interference (SQUID) devices placed near the scalp, within a shielded room to prevent magnetic interference. MEG provides a spatial resolution of 1–2 mm and a response time of milliseconds, but requires a bulky device with high operating costs.

A new type of detector, the “optically pumped magnetometer” (OPM), improves MEG by measuring the brain’s magnetic field at room temperature. OPM uses a small cell filled with an alkali atom vapour. A laser diode tuned to a specific quantum transition optically pumps the vapour, which aligns the atomic magnetic moments. This magnetization interacts with the brain’s magnetic field to change the opacity of the vapour as determined by a detector, which makes it possible to measure the magnetic field.
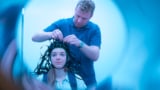
Quantum physics gives brain-sensing MEG scanners a boost
Earlier this year, UK-based firm Cerca Magnetics won an award in quantum innovation for the development of its OPM-MEG wearable brain scanner. This comprises 50 LEGO block-sized units mounted on a whole-head helmet to cover the brain. The prototype wearable OPM-MEG BCI allows neural diagnosis as a subject moves. With its high spatial and temporal resolutions, it could possibly control external devices.
Listening to the brain
Ultrasound technology is widely used as a portable non-invasive method to image bodily structures, including red blood cells, as they reflect high-frequency sound waves. In the last decade, the technology has developed to the point that “fast functional ultrasound” (fUS) can use Doppler measurements of the brain’s blood flow to identify active neurons. In fUS, probes generate ultrasonic plane waves and gather data over hundreds of channels. A computer then synthetically focuses the waves and analyses the data to rapidly produce high-resolution images of brain function. Studies in non-human primates show that fUS operating through a minimally invasive port in the cranium could support a BCI that tracks the neural impulses representing bodily motion (Neuroscience 474 110).
Ultrasound also serves in transcranial ultrasound stimulation (TUS), a method to modulate neural behaviour that can be targeted to within a few cubic millimetres within the brain. After extensive animal studies, some human trials suggest that TUS can treat neurological or psychiatric problems such as pain and depression.
The future of non-invasive BCIs
Complementing and perhaps someday replacing implants, other physical methods can access the brain with minimal invasiveness, enabling safer, cheaper and wider medical use of BCIs. Andrew Jackson, a physicist-turned-neuroscientist at Newcastle University, UK, says that, when it comes to recording the brain, the most exciting technology at the moment is wearable OPM-MEG. “It’s interesting physics too!” he adds, noting the value of ultrasound for brain stimulation. Jackson warns, though, that none of these non-invasive technologies yet has the spatial resolution that you can get with implants. Much remains to be done for clinical use, and perhaps beyond it.

If non-invasive BCIs eliminate surgical risk, healthy individuals could be motivated to use them for real or perceived mental augmentation. Noted neuroscientist Kristof Koch has related how “awesome” it would be to have a safe BCI that links brains to computers so people could download information directly into their brains.
In 2021 San Francisco start-up MindPortal raised $5m to develop a headband for mental control of a virtual-reality game. It uses proprietary technology, perhaps a fast-NIR method. In another application, transcranial direct current stimulation (tDCS) devices are readily available at modest prices. These apply milliamp electric currents to the skull that supposedly improve cognition.
Seeing the rise of consumer neurotech, neuroethicists point to the harm that could come without effective oversight and regulation – which would also need to consider issues such as privacy and mind control. In developing non-invasive BCIs, researchers are hugely advancing brain research and treatment, helping to restore the independence of severely disabled individuals. At the same time, researchers should be aware of the many ethical quandaries that these devices raise, beyond the lab and clinic.
- SEO Powered Content & PR Distribution. Get Amplified Today.
- PlatoData.Network Vertical Generative Ai. Empower Yourself. Access Here.
- PlatoAiStream. Web3 Intelligence. Knowledge Amplified. Access Here.
- PlatoESG. Carbon, CleanTech, Energy, Environment, Solar, Waste Management. Access Here.
- PlatoHealth. Biotech and Clinical Trials Intelligence. Access Here.
- Source: https://physicsworld.com/a/plug-me-in-the-physics-of-brain-computer-interfaces/
- :has
- :is
- :not
- :where
- 09
- 1
- 10
- 100
- 160
- 1998
- 2015
- 2019
- 2021
- 2022
- 25
- 30
- 3d
- 400
- 50
- 700
- 80
- 90
- a
- abilities
- absorbs
- access
- accident
- accurately
- across
- activated
- active
- activities
- activity
- actually
- adapting
- Adds
- administration
- advance
- advancing
- affect
- After
- agency
- Aligns
- Alkali
- All
- allow
- allowed
- Allowing
- allows
- also
- among
- an
- analyse
- analyses
- and
- animal
- announced
- Another
- answer
- any
- apart
- Application
- applications
- applied
- Apply
- approach
- approaches
- appropriate
- approval
- approved
- ARE
- areas
- ARM
- Array
- arrival
- artificial
- AS
- astounding
- At
- atom
- attempting
- available
- award
- aware
- away
- background
- basis
- bci
- BE
- became
- because
- been
- behaviour
- being
- believes
- below
- benefit
- Berger
- bespoke
- Better
- between
- Beyond
- Billion
- Biophysics
- BlackRock
- blood
- Blue
- body
- Box
- Brain
- Brain activity
- brains
- breakthrough
- BRIDGE
- BSC
- but
- by
- called
- CAN
- Can Get
- candidates
- cannot
- car
- carbon
- carbon nanotubes
- carried
- Cause
- cell
- Cells
- cellular
- Century
- cerebral
- challenge
- Chance
- change
- Changes
- channels
- cheaper
- child
- Choose
- circulating
- clear
- click
- clinic
- Clinical
- clinicians
- cognition
- cognitive
- colleagues
- collect
- combination
- combined
- come
- comes
- Common
- commonly
- communicate
- community
- company
- compared
- complex
- comprises
- computer
- computer screen
- computer-generated
- computers
- computing
- concepts
- conditions
- conducting
- Configuration
- Consider
- considered
- consumer
- contacts
- continue
- control
- controlling
- cortex
- Costs
- could
- cover
- covered
- covers
- create
- created
- crucial
- Current
- Current state
- Currently
- cutting-edge
- daily
- damage
- dangers
- data
- decade
- deep
- deeper
- Delayed
- deliver
- delivering
- demanding
- Dementia
- demonstrated
- density
- Department
- Design
- designed
- details
- detect
- detected
- Determine
- determined
- develop
- developed
- developing
- Development
- developments
- device
- Devices
- diagnose
- diagnosis
- difference
- different
- difficult
- direct
- directly
- disabled
- displayed
- distance
- does
- doing
- done
- Dont
- down
- download
- draws
- drug
- Drugs
- dubbed
- due
- each
- Early
- Effective
- efforts
- either
- Electric
- Electronics
- eliminate
- Elon
- Elon Musk
- enabling
- Engineering
- enhance
- Enhances
- establish
- established
- Ether (ETH)
- ethical
- Even
- eventually
- examine
- example
- examples
- excited
- exciting
- executed
- existing
- expensive
- experimental
- experiments
- exposed
- extensive
- external
- extract
- extremely
- fact
- fails
- FAST
- Fat
- fda
- few
- Fiction
- field
- Fields
- filled
- finding
- Firm
- First
- first time
- fit
- flexible
- flow
- focused
- focuses
- following
- food
- Food and Drug Administration
- Food and Drug Administration (FDA)
- For
- form
- forms
- forthcoming
- four
- Francisco
- from
- full
- fully
- function
- functional
- functions
- fundamental
- further
- future
- game
- Games
- gaming
- gather
- generate
- generated
- German
- get
- gives
- good
- Group
- Group’s
- had
- hand
- Hands
- harm
- Have
- he
- head
- Headset
- healthy
- Held
- help
- helped
- helping
- her
- High
- High-Frequency
- high-resolution
- higher
- him
- his
- hold
- How
- HTTPS
- Hugely
- human
- Hundreds
- idea
- identify
- image
- images
- imagine
- immune
- Impact
- implications
- important
- improve
- improvements
- improves
- in
- include
- Including
- increased
- Increases
- independence
- individual
- individuals
- Infections
- inflammation
- inflict
- information
- initial
- Innovation
- insight
- installation
- instance
- instead
- Institute
- interact
- interaction
- interacts
- interesting
- Interface
- interfaces
- interference
- Internet
- into
- invasive
- investigate
- Ionic
- issue
- issues
- IT
- ITS
- Jackson
- jpg
- Keep
- Knowing
- known
- Koch
- lab
- Label
- large
- laser
- Last
- layer
- learned
- left
- less
- Level
- lie
- Life
- light
- like
- limitations
- Limited
- LINK
- links
- Lives
- Long
- long-term
- lost
- Ltd
- machinery
- Magnetic field
- make
- MAKES
- Making
- man
- manufacturing
- many
- map
- mapping
- massachusetts
- Massachusetts Institute of technology
- Match
- material
- materials
- max-width
- maximum
- May..
- me
- meaning
- means
- measure
- measured
- measurement
- measurements
- measuring
- mechanical
- mechanism
- medical
- Medical physics
- mental
- mentioned
- method
- methods
- might
- millions
- milliseconds
- mind
- minimal
- minimize
- missing
- MIT
- model
- modest
- modify
- Modules
- moment
- Moments
- mood
- more
- most
- motion
- motivated
- move
- moved
- moves
- moving
- MRI
- much
- Musk
- Navigate
- Near
- nearly
- Need
- needed
- Neural
- neuralink
- neurological
- Neurons
- Neuroscience
- New
- NIH
- None
- notable
- noted
- noting
- now
- obtained
- of
- often
- on
- once
- ONE
- only
- open
- operate
- operating
- optical
- or
- organic
- Other
- our
- out
- over
- Oversight
- Pain
- parts
- patient
- patients
- People
- per
- perceived
- perfectly
- performance
- perhaps
- permanently
- person
- phd
- photographer
- Photons
- physical
- Physics
- Physics World
- piece
- pink
- Place
- placed
- plane
- platinum
- plato
- Plato Data Intelligence
- PlatoData
- Play
- plug
- Point
- Polymers
- poor
- portable
- possession
- possible
- possibly
- potential
- potentially
- power
- prevent
- Prices
- principles
- privacy
- probe
- Problem
- problems
- process
- produce
- Produced
- Product
- promise
- promising
- properties
- proprietary
- protons
- prototype
- provides
- providing
- published
- pumps
- quality
- Quantum
- Questions
- raise
- raised
- rapidly
- rather
- ratio
- readily
- real
- real-time
- realized
- realms
- recent
- record
- recorded
- recording
- Red
- reduce
- reduces
- reflect
- regions
- Regulation
- related
- remains
- REPEATEDLY
- report
- representing
- require
- requires
- research
- researchers
- Resolution
- resonance
- response
- response times
- responses
- responsible
- restore
- Results
- rigid
- rigorous
- Rise
- Risk
- risks
- Risky
- robot
- Robotic
- robotics
- Room
- roughly
- runs
- Russia
- s
- safe
- safely
- safer
- same
- San
- San Francisco
- say
- says
- Science
- Scientist
- Screen
- Second
- seconds
- see
- selected
- send
- sensitive
- serious
- serves
- several
- severe
- severely
- she
- Short
- should
- show
- showed
- shown
- Sight
- signals
- Silicon
- since
- single
- Sites
- Size
- slow
- small
- So
- Society
- Soft
- solely
- Solutions
- some
- someday
- Sound
- Sources
- Spatial
- speak
- specific
- Spectroscopy
- Spectrum
- speed
- SPELL
- square
- Stability
- standard
- stanford
- Stanford university
- Start-up
- State
- Step
- Still
- strong
- structures
- studies
- studios
- Study
- subject
- such
- suffering
- suggest
- suitable
- support
- Surface
- Surgery
- surgical
- Symptoms
- Synapses
- synthetically
- tailored
- takes
- tapping
- targeted
- technically
- technique
- Technologies
- Technology
- tens
- test
- text
- than
- thanks
- that
- The
- the world
- their
- Them
- then
- There.
- thereby
- These
- they
- this
- this year
- those
- though?
- thought
- thousand
- thousands
- Through
- thumbnail
- time
- times
- tissue
- to
- today
- too
- tool
- toward
- trace
- tracks
- trained
- trans
- transition
- translate
- treat
- treatment
- trial
- trials
- tried
- trigger
- trouble
- true
- truly
- tumours
- TURN
- Turned
- two
- type
- types
- typically
- Uk
- Ultrasonic
- ultrasound
- unable
- under
- understood
- units
- university
- us
- US Food
- use
- used
- uses
- using
- utah
- Valuable
- value
- variations
- various
- varying
- version
- Vessel
- vessels
- via
- Video
- video games
- Virtual reality
- Warns
- was
- Water
- wavelengths
- waves
- ways
- wearable
- WELL
- were
- whatever
- when
- which
- while
- WHO
- wide
- widely
- wider
- will
- wireless
- with
- within
- without
- Won
- words
- Work
- worked
- working
- works
- world
- worldwide
- would
- writing
- year
- years
- yet
- you
- young
- Your
- zephyrnet