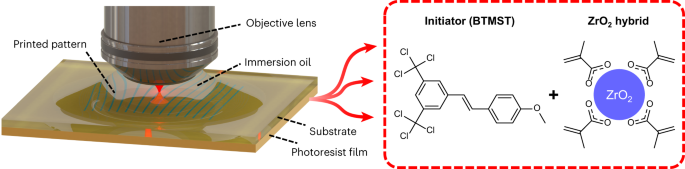
Erkal, J. L. et al. 3D printed microfluidic devices with integrated versatile and reusable electrodes. Lab Chip 14, 2023–2032 (2014).
Huang, T. Y. et al. 3D printed microtransporters: compound micromachines for spatiotemporally controlled delivery of therapeutic agents. Adv. Mater. 27, 6644–6650 (2015).
von Freymann, G. et al. Three-dimensional nanostructures for photonics. Adv. Funct. Mater. 20, 1038–1052 (2010).
Xiong, W. et al. Laser-directed assembly of aligned carbon nanotubes in three dimensions for multifunctional device fabrication. Adv. Mater. 28, 2002–2009 (2016).
Zhang, W. et al. 3D printed micro-electrochemical energy storage devices: from design to integration. Adv. Funct. Mater. 31, 2104909 (2021).
Wei, T. S., Ahn, B. Y., Grotto, J. & Lewis, J. A. 3D printing of customized Li-ion batteries with thick electrodes. Adv. Mater. 30, 1703027 (2018).
Symes, M. D. et al. Integrated 3D-printed reactionware for chemical synthesis and analysis. Nat. Chem. 4, 349–354 (2012).
Derby, B. Printing and prototyping of tissues and scaffolds. Science 338, 921–926 (2012).
Lee, A. et al. 3D bioprinting of collagen to rebuild components of the human heart. Science 365, 482–487 (2019).
Kawata, S., Sun, H. B., Tanaka, T. & Takada, K. Finer features for functional microdevices. Nature 412, 697–698 (2001).
Regehly, M. et al. Xolography for linear volumetric 3D printing. Nature 588, 620–624 (2020).
Guo, L. J. Nanoimprint lithography: methods and material requirements. Adv. Mater. 19, 495–513 (2007).
Tumbleston, J. R. et al. Continuous liquid interface production of 3D objects. Science 347, 1349–1352 (2015).
Tseng, A. A., Notargiacomo, A. & Chen, T. P. Nanofabrication by scanning probe microscope lithography: a review. J. Vac. Sci. Technol. B 23, 877–894 (2005).
Arnoux, C. et al. Polymerization photoinitiators with near-resonance enhanced two-photon absorption cross-section: toward high-resolution photoresist with improved sensitivity. Macromolecules 53, 9264–9278 (2020).
Gan, Z., Cao, Y., Evans, R. A. & Gu, M. Three-dimensional deep sub-diffraction optical beam lithography with 9 nm feature size. Nat. Commun. 4, 2061 (2013).
Jin, F. et al. λ/30 inorganic features achieved by multi-photon 3D lithography. Nat. Commun. 13, 1357 (2022).
Portela, C. M. et al. Supersonic impact resilience of nanoarchitected carbon. Nat. Mater. 20, 1491–1497 (2021).
Geng, Q., Wang, D., Chen, P. & Chen, S. C. Ultrafast multi-focus 3-D nano-fabrication based on two-photon polymerization. Nat. Commun. 10, 2179 (2019).
Oakdale, J. S. et al. Direct laser writing of low-density interdigitated foams for plasma drive shaping. Adv. Funct. Mater. 27, 1702425 (2017).
Fischer, J. et al. Three-dimensional multi-photon direct laser writing with variable repetition rate. Opt. Express 21, 26244–26260 (2013).
Meza, L. R., Das, S. & Greer, J. R. Strong, lightweight, and recoverable three-dimensional ceramic nanolattices. Science 345, 1322–1326 (2014).
Malinauskas, M., Zukauskas, A., Bickauskaite, G., Gadonas, R. & Juodkazis, S. Mechanisms of three-dimensional structuring of photo-polymers by tightly focussed femtosecond laser pulses. Opt. Express 18, 10209–10221 (2010).
Shaw, L. A. et al. Scanning two-photon continuous flow lithography for synthesis of high-resolution 3D microparticles. Opt. Express 26, 13543–13548 (2018).
Ito, H. Chemical amplification resists for microlithography. Adv. Polym. Sci. 172, 37–245 (2005).
Ito, H. Chemical amplification resists: inception, implementation in device manufacture, and new developments. J. Polym. Sci. A 41, 3863–3870 (2003).
Ito, H. Chemical amplification resists: History and development within IBM. IBM J. Res. Dev. 41, 69–80 (1997).
Bourzac, K. A giant bid to etch tiny circuits. Nature 487, 419 (2012).
Lithography roadmap on track. Nat. Photon. 4, 20 (2010).
Totzeck, M., Ulrich, W., Göhnermeier, A. & Kaiser, W. Pushing deep ultraviolet lithography to its limits. Nat. Photon. 1, 629–631 (2007).
Trikeriotis, M. et al. Nanoparticle photoresists from HfO2 and ZrO2 for EUV patterning. J. Photopolym. Sci. Technol. 25, 583–586 (2012).
Jiang, J., Chakrabarty, S., Yu, M. & Ober, C. K. Metal oxide nanoparticle photoresists for EUV patterning. J. Photopolym. Sci. Technol. 27, 663–666 (2014).
Xu, H. et al. Metal-organic framework-inspired metal-containing clusters for high-resolution patterning. Chem. Mater. 30, 4124–4133 (2018).
Tanaka, H., Matsumoto, A., Akinaga, K., Takahashi, A. & Okada, T. Comparative study on emission characteristics of extreme ultraviolet radiation from CO2 and Nd:YAG laser-produced tin plasmas. Appl. Phys. Lett. 87, 041503 (2005).
Service, R. F. Optical lithography goes to extremes-and beyond. Science 293, 785–786 (2001).
The shrinking chip. Nat. Photonics 3, 485 (2009).
Xu, H., Kosma, V., Giannelis, E. P. & Ober, C. K. In pursuit of Moore’s Law: polymer chemistry in action. Polym. J. 50, 45–55 (2018).
Rayleigh, L. On the theory of optical images, with special reference to the microscope. J. R. Microsc. Soc. 42, 167–195 (2011).
Wagner, C. & Harned, N. Lithography gets extreme. Nat. Photon. 4, 24–26 (2010).
Sanders, D. P. Advances in patterning materials for 193 nm immersion lithography. Chem. Rev. 110, 321–360 (2010).
Pohlers, G., Scaiano, J. C., Step, E. & Sinta, R. Ionic vs free radical pathways in the direct and sensitized photochemistry of 2-(4′-methoxynaphthyl)-4,6-bis(trichloromethyl)-1,3,5-triazine: relevance for photoacid generation. J. Am. Chem. Soc. 121, 6167–6175 (1999).
Pohlers, G., Scaiano, J. C., Sinta, R., Brainard, R. & Pai, D. Mechanistic studies of photoacid generation from substituted 4,6-bis(trichloromethyl)-1,3,5-triazines. Chem. Mater. 9, 1353–1361 (1997).
Ligon, S. C., Husar, B., Wutzel, H., Holman, R. & Liska, R. Strategies to reduce oxygen inhibition in photoinduced polymerization. Chem. Rev. 114, 557–589 (2014).
Lu, W. E., Dong, X. Z., Chen, W. Q., Zhao, Z. S. & Duan, X. M. Novel photoinitiator with a radical quenching moiety for confining radical diffusion in two-photon induced photopolymerization. J. Mater. Chem. 21, 5650–5659 (2011).
Sheik-Bahae, M., Said, A. A., Wei, T. H., Hagan, D. J. & Van Stryland, E. W. Sensitive measurement of optical nonlinearities using a single beam. IEEE J. Quantum Electron. 26, 760–769 (1990).
Sheik-Bahae, M., Said, A. A. & Van Stryland, E. W. High-sensitivity, single-beam n2 measurements. Opt. Lett. 14, 955–957 (1989).
Buckingham, A. D., Fowler, P. W. & Hutson, J. M. Theoretical studies of van der Waals molecules and intermolecular forces. Chem. Rev. 88, 963–988 (1988).
Berland, K. et al. Van der Waals forces in density functional theory: a review of the vdW-DF method. Rep. Prog. Phys. 78, 066501 (2015).
Ouyang, W. et al. Ultrafast 3D nanofabrication via digital holography. Nat. Commun. 14, 1716 (2023).
Saha, S. K. et al. Scalable submicrometer additive manufacturing. Science 366, 105–109 (2019).
Sheng, L. et al. Suppressing electrolyte-lithium metal reactivity via Li+-desolvation in uniform nano-porous separator. Nat. Commun. 13, 172 (2022).
Hohenberg, P. & Kohn, W. Inhomogeneous electron gas. Phys. Rev. B 136, B864–B871 (1964).
Kohn, W. & Sham, L. J. Self-consistent equations including exchange and correlation effects. Phys. Rev. 140, 1133–1138 (1965).
Andzelm, J., Kolmel, C. & Klamt, A. Incorporation of solvent effects into density functional calculations of molecular energies and geometries. J. Chem. Phys. 103, 9312–9320 (1995).
Klamt, A., Jonas, V., Burger, T. & Lohrenz, J. C. W. Refinement and parametrization of COSMO-RS. J. Phys. Chem. A 102, 5074–5085 (1998).
Mullins, E. et al. Sigma-profile database for using COSMO-based thermodynamic methods. Ind. Eng. Chem. Res. 45, 4389–4415 (2006).
Mullins, E., Liu, Y. A., Ghaderi, A. & Fast, S. D. Sigma profile database for predicting solid solubility in pure and mixed solvent mixtures for organic pharmacological compounds with COSMO-based thermodynamic methods. Ind. Eng. Chem. Res. 47, 1707–1725 (2008).
Delley, B. An all-electron numerical method for solving the local density functional for polyatomic molecules. J. Chem. Phys. 92, 508–517 (1990).
Delley, B. From molecules to solids with the DMol3 approach. J. Chem. Phys. 113, 7756–7764 (2000).
Zhao, Y. & Truhlar, D. G. A new local density functional for main-group thermochemistry, transition metal bonding, thermochemical kinetics, and noncovalent interactions. J. Chem. Phys. 125, 194101 (2006).
Perdew, J. P., Burke, K. & Ernzerhof, M. Generalized gradient approximation made simple. Phys. Rev. Lett. 77, 3865–3868 (1996).
Adamo, C. & Barone, V. Toward reliable density functional methods without adjustable parameters: the PBE0 model. J. Chem. Phys. 110, 6158–6170 (1999).
Ernzerhof, M. & Scuseria, G. E. Assessment of the Perdew–Burke–Ernzerhof exchange-correlation functional. J. Chem. Phys. 110, 5029–5036 (1999).
Weigend, F. & Ahlrichs, R. Balanced basis sets of split valence, triple zeta valence and quadruple zeta valence quality for H to Rn: design and assessment of accuracy. Phys. Chem. Chem. Phys. 7, 3297–3305 (2005).
Weigend, F. Accurate Coulomb-fitting basis sets for H to Rn. Phys. Chem. Chem. Phys. 8, 1057–1065 (2006).
Marenich, A. V., Cramer, C. J. & Truhlar, D. G. Universal solvation model based on solute electron density and on a continuum model of the solvent defined by the bulk dielectric constant and atomic surface tensions. J. Phys. Chem. B 113, 6378–6396 (2009).
Frisch, M. J. et al. Gaussian 16 Revision C (Gaussian, 2016).
Lu, T. & Chen, F. Multiwfn: a multifunctional wavefunction analyzer. J. Comput. Chem. 33, 580–592 (2012).
- SEO Powered Content & PR Distribution. Get Amplified Today.
- PlatoData.Network Vertical Generative Ai. Empower Yourself. Access Here.
- PlatoAiStream. Web3 Intelligence. Knowledge Amplified. Access Here.
- PlatoESG. Carbon, CleanTech, Energy, Environment, Solar, Waste Management. Access Here.
- PlatoHealth. Biotech and Clinical Trials Intelligence. Access Here.
- Source: https://www.nature.com/articles/s41565-023-01517-w
- ][p
- 1
- 10
- 11
- 12
- 13
- 14
- 15%
- 16
- 17
- 19
- 1995
- 1996
- 1998
- 1999
- 20
- 2000
- 2001
- 2005
- 2006
- 2008
- 2009
- 2010
- 2011
- 2012
- 2013
- 2014
- 2015
- 2016
- 2017
- 2018
- 2019
- 2020
- 2021
- 2022
- 2023
- 22
- 23
- 24
- 25
- 26
- 27
- 28
- 29
- 30
- 31
- 32
- 33
- 36
- 39
- 3d
- 3D Printing
- 40
- 41
- 46
- 49
- 50
- 51
- 54
- 60
- 65
- 66
- 67
- 7
- 75
- 77
- 8
- 9
- a
- accuracy
- accurate
- achieved
- Action
- additive
- additive manufacturing
- adjustable
- advances
- agents
- AL
- aligned
- am
- Amplification
- an
- analysis
- and
- approach
- article
- Assembly
- assessment
- b
- balanced
- based
- basis
- batteries
- Beam
- Beyond
- bid
- burger
- by
- calculations
- carbon
- carbon nanotubes
- characteristics
- chemical
- chemistry
- chen
- chip
- click
- components
- Compound
- constant
- continuous
- Continuum
- controlled
- Correlation
- customized
- Database
- deep
- defined
- delivery
- density
- Design
- Dev
- Development
- developments
- device
- Devices
- Diffusion
- digital
- dimensions
- direct
- drive
- e
- E&T
- effects
- emission
- energy
- enhanced
- equations
- Ether (ETH)
- exchange
- extreme
- FAST
- Feature
- Features
- flow
- focussed
- For
- Forces
- Free
- from
- functional
- GAS
- generation
- giant
- Goes
- Heart
- high-resolution
- history
- holography
- http
- HTTPS
- human
- IBM
- images
- immersion
- Impact
- implementation
- improved
- in
- inception
- Including
- integrated
- integration
- interactions
- Interface
- into
- Ionic
- ITS
- laser
- Law
- Lewis
- lightweight
- limits
- LINK
- Liquid
- local
- made
- manufacturing
- material
- materials
- measurement
- measurements
- mechanisms
- metal
- method
- methods
- Microscope
- mixed
- model
- molecular
- nanotechnology
- Nature
- New
- novel
- numerical
- objects
- of
- on
- optical
- organic
- Oxygen
- parameters
- pathways
- Plasma
- plato
- Plato Data Intelligence
- PlatoData
- polymer
- predicting
- printing
- probe
- Production
- Profile
- prototyping
- pursuit
- Pushing
- quality
- Quantum
- R
- Radiation
- radical
- Rate
- reduce
- reference
- relevance
- reliable
- Requirements
- resilience
- reusable
- review
- roadmap
- s
- Said
- scalable
- scanning
- Scholar
- SCI
- sensitive
- Sensitivity
- Sets
- shaping
- Sigma
- Simple
- single
- Size
- solid
- Solving
- special
- split
- Step
- storage
- strategies
- strong
- structuring
- studies
- Study
- Sun
- supersonic
- suppressing
- Surface
- T
- tensions
- The
- theoretical
- theory
- Therapeutic
- three
- three-dimensional
- tightly
- tissues
- to
- toward
- track
- transition
- Triple
- Universal
- using
- variable
- versatile
- via
- volumetric
- vs
- W
- wang
- with
- within
- without
- writing
- X
- zephyrnet
- Zeta
- Zhao