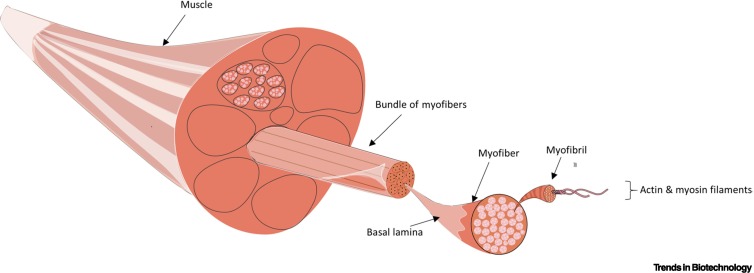
Engineered skeletal muscle units for repair of volumetric muscle loss in the tibialis anterior muscle of a rat.
Tissue Eng. A. 2014; 20: 2920-2930
Engineering muscle constructs for the creation of functional engineered musculoskeletal tissue.
Regen. Med. 2014; 9: 89-100
Functional analysis of limb recovery following autograft treatment of volumetric muscle loss in the quadriceps femoris.
J. Biomech. 2014; 47: 2013-2021
The factors present in regenerating muscles impact bone marrow-derived mesenchymal stromal/stem cell fusion with myoblasts.
Stem Cell Res Ther. 2019; 10: 1-17
Advances in biomaterials for skeletal muscle engineering and obstacles still to overcome.
Mater. Today Bio. 2020; 7100069
Biomimetic scaffolds for regeneration of volumetric muscle loss in skeletal muscle injuries.
Acta Biomater. 2015; 25: 2-15
Biomaterial-based delivery for skeletal muscle repair.
Adv. Drug Deliv. Rev. 2015; 84: 188-197
Design, evaluation, and application of engineered skeletal muscle.
Methods. 2016; 99: 81-90
3D in vitro models of skeletal muscle: myopshere, myobundle and bioprinted muscle construct.
Vet. Res. 2021; 52: 1-12
Transcriptome-scale similarities between mouse and human skeletal muscles with normal and myopathic phenotypes.
BMC Musculoskelet. Disord. 2006; 7: 1-9
Anatomical comparison across heads, fore- and hindlimbs in mammals using network models.
J. Anat. 2021; 239: 12-31
Terrestrial locomotion energy costs vary considerably between species: no evidence that this is explained by rate of leg force production or ecology.
Sci. Reports. 2019; 9: 1-13
Assessment of muscle mass and strength in mice.
Bonekey Rep. 2015; 4: 732
Regeneration of skeletal muscle using in situ tissue engineering on an acellular collagen sponge scaffold in a rabbit model.
ASAIO J. 2007; 53: 506-513
Autologous minced muscle grafts improve muscle strength in a porcine model of volumetric muscle loss injury.
J. Orthop. Trauma. 2016; 30: e396-e403
Biologic scaffold remodeling in a dog model of complex musculoskeletal injury.
J. Surg. Res. 2012; 176: 490-502
The Dunkin Hartley guinea pig is a model of primary osteoarthritis that also exhibits early onset myofiber remodeling that resembles human musculoskeletal aging.
Front. Physiol. 2020; 11: 1412
Comparison of quadriceps and hamstring muscle activity during an isometric squat between strength-matched men and women.
J. Sports Sci. Med. 2019; 18: 101
Comparative study of injury models for studying muscle regeneration in mice.
PLoS ONE. 2016; 11e0147198
Freeze injury of the tibialis anterior muscle.
Methods Mol. Biol. 2016; 1460: 33-41
Injectable laminin-biofunctionalized gellan gum hydrogels loaded with myoblasts for skeletal muscle regeneration.
Acta Biomater. 2022; 143: 282-294
Gold and gold-silver alloy nanoparticles enhance the myogenic differentiation of myoblasts through p38 MAPK signaling pathway and promote in vivo skeletal muscle regeneration.
Biomaterials. 2018; 175: 19-29
Degradable conductive self-healing hydrogels based on dextran-graft-tetraaniline and N-carboxyethyl chitosan as injectable carriers for myoblast cell therapy and muscle regeneration.
Acta Biomater. 2019; 84: 180-193
Muscle stem cell intramuscular delivery within hyaluronan methylcellulose improves engraftment efficiency and dispersion.
Biomaterials. 2018; 173: 34-46
In vivo tissue engineering of functional skeletal muscle by freshly isolated satellite cells embedded in a photopolymerizable hydrogel.
FASEB J. 2011; 25: 2296-2304
Adipose-derived stem/stromal cells on electrospun fibrin microfiber bundles enable moderate muscle reconstruction in a volumetric muscle loss model.
Cell Transplant. 2018; 27: 1644-1656
Treatment of volumetric muscle loss in mice using nanofibrillar scaffolds enhances vascular organization and integration.
Commun. Biol. 2019; 2: 170
Engineering functional and histological regeneration of vascularized skeletal muscle.
Biomaterials. 2018; 164: 70-79
Nanopatterned muscle cell patches for enhanced myogenesis and dystrophin expression in a mouse model of muscular dystrophy.
Biomaterials. 2014; 35: 1478-1486
Engineering vascularized skeletal muscle tissue.
Nat. Biotechnol. 2005; 23: 879-884
Therapeutic effects of mouse adipose-derived stem cells and losartan in the skeletal muscle of injured mdx mice.
Cell Transplant. 2015; 24: 939-953
Humanizing the mdx mouse model of DMD: the long and the short of it.
npj Regen. Med. 2018; 3: 1-11
Muscle injuries and strategies for improving their repair.
J. Exp. Orthop. 2016; 3: 15
Combination therapy of human adipose-derived stem cells and basic fibroblast growth factor hydrogel in muscle regeneration.
Biomaterials. 2013; 34: 6037-6045
Skeletal muscle derived stem cells microintegrated into a biodegradable elastomer for reconstruction of the abdominal wall.
Biomaterials. 2017; 113: 31-41
Muscular tissue engineering: capillary-incorporated hybrid muscular tissues in vivo tissue culture.
Cell Transplant. 1998; 7: 435-442
Injectable thermosensitive chitosan/b-glycerophosphate/collagen hydrogel maintains the plasticity of skeletal muscle satellite cells and supports their in vivo viability.
Cell Biol. Int. 2013; 37: 977-987
Autologous transplantation of adipose-derived stem cells improves functional recovery of skeletal muscle without direct participation in new myofiber formation.
Stem Cell Res Ther. 2018; 9: 195
Microfluidic-enhanced 3D bioprinting of aligned myoblast-laden hydrogels leads to functionally organized myofibers in vitro and in vivo.
Biomaterials. 2017; 131: 98-110
The effect of in vitro formation of acetylcholine receptor (AChR) clusters in engineered muscle fibers on subsequent innervation of constructs in vivo.
Biomaterials. 2013; 34: 3246-3255
Tissue engineering of injectable muscle: three-dimensional myoblast-fibrin injection in the syngeneic rat animal model.
Plast. Reconstr. Surg. 2006; 118: 1113-1121
Biomimetic engineered muscle with capacity for vascular integration and functional maturation in vivo.
Proc. Natl. Acad. Sci. U. S. A. 2014; 111: 5508-5513
Laminin mimetic peptide nanofibers regenerate acute muscle defect.
Acta Biomater. 2017; 60: 190-200
Synthetic niche to modulate regenerative potential of MSCs and enhance skeletal muscle regeneration.
Biomaterials. 2016; 99: 95-108
Endogenous electric signaling as a blueprint for conductive materials in tissue engineering.
Bioelectricity. 2021; 3: 27-41
Conductive biomaterials for muscle tissue engineering.
Biomaterials. 2020; 229119584
Electric phenomenon: a disregarded tool in tissue engineering and regenerative medicine.
Trends Biotechnol. 2020; 38: 24-49
Electroactive gellan gum/polyaniline spongy-like hydrogels.
ACS Biomater. Sci. Eng. 2018; 4: 1779-1787
Biomimetic elastomeric, conductive and biodegradable polycitrate-based nanocomposites for guiding myogenic differentiation and skeletal muscle regeneration.
Biomaterials. 2018; 157: 40-50
The effect of VEGF on the myogenic differentiation of adipose tissue derived stem cells within thermosensitive hydrogel matrices.
Biomaterials. 2010; 31: 1213-1218
Losartan administration reduces fibrosis but hinders functional recovery after volumetric muscle loss injury.
J. Appl. Physiol. 2014; 117: 1120-1131
Losartan enhances the success of myoblast transplantation.
Cell Transplant. 2012; 21: 139-152
Losartan improves adipose tissue-derived stem cell niche by inhibiting transforming growth factor-β and fibrosis in skeletal muscle injury.
Cell Transplant. 2012; 21: 2407-2424
Stem cells and growth factors-based delivery approaches for chronic wound repair and regeneration: a promise to heal from within.
Life Sci. 2021; 268118932
Isolation and purification of satellite cells for skeletal muscle tissue engineering.
J. Regen. Med. 2014; 3: 117
Myoblast-conditioned media improve regeneration and revascularization of ischemic muscles in diabetic mice.
Stem Cell Res Ther. 2015; 6: 61
FACS-purified myoblasts producing controlled VEGF levels induce safe and stable angiogenesis in chronic hind limb ischemia.
J. Cell. Mol. Med. 2012; 16: 107-117
Mesenchymal stem cells for regenerative medicine for Duchenne muscular dystrophy.
in: Gaina G. Muscular Dystrophy: Research Updates and Therapeutic Strategies. IntechOpen, 2020https://doi.org/10.5772/intechopen.92824
In vitro differentiation of rat mesenchymal stem cells into skeletal muscle cells induced by myoblast differentiation factor and 5-azacytidine.
Chinese J. Reparative Reconstr. Surg. 2007; 21: 1371-1375
Review of the adipose derived stem cell secretome.
Biochimie. 2013; 95: 2222-2228
Adipose tissue derived stem cells secretome: soluble factors and their roles in regenerative medicine.
Curr. Stem Cell Res. Ther. 2010; 5: 103-110
Human ES- and iPS-derived myogenic progenitors restore DYSTROPHIN and improve contractility upon transplantation in dystrophic mice.
Cell Stem Cell. 2012; 10: 610-619
Engineering human pluripotent stem cells into a functional skeletal muscle tissue.
Nat. Commun. 2018; 9: 126
Skeletal muscle generated from induced pluripotent stem cells – induction and application.
World J. Stem Cells. 2017; 9: 8997
Global trends in clinical trials involving pluripotent stem cells: a systematic multi-database analysis.
NPJ Regen. Med. 2020; 5: 15
The pathophysiology of skeletal muscle ischemia and the reperfusion syndrome: a review.
Cardiovasc. Surg. 2002; 10: 620-630
The role of endothelial cells in myofiber differentiation and the vascularization and innervation of bioengineered muscle tissue in vivo.
Biomaterials. 2013; 34: 140-149
Endothelial differentiation of mesenchymal stromal cells.
PLoS ONE. 2012; 7e46842
Isolation of endothelial cells from murine tissue.
J. Immunol. Methods. 2000; 244: 205-215
Results of a triple blind clinical study of myoblast transplantations without immunosuppressive treatment in young boys with Duchenne muscular dystrophy.
Cell Transplant. 1993; 2: 99-112
Myoblast transplantation between monozygotic twin girl carriers of Duchenne muscular dystrophy.
Neuromuscul. Disord. 1993; 3: 583-592
Effect of needle diameter and flow rate on rat and human mesenchymal stromal cell characterization and viability.
Tissue Eng. C Methods. 2010; 16: 989-997
Devices for cell transplantation into the central nervous system: design considerations and emerging technologies.
Surg. Neurol. Int. 2013; 4: 22
Transplantation of human umbilical cord-derived mesenchymal stems cells for the treatment of Becker muscular dystrophy in affected pedigree members.
Int. J. Mol. Med. 2015; 35: 1051-1057
Efficacy of stem cell therapy in ambulatory and nonambulatory children with Duchenne muscular dystrophy – Phase I-II.
Degener. Neurol. Neuromuscul. Dis. 2018; 8: 63-77
Immunomodulatory placental-expanded, mesenchymal stromal cells improve muscle function following hip arthroplasty.
J. Cachexia. Sarcopenia Muscle. 2018; 9: 880-897
The formation of skeletal muscle: from somite to limb.
J. Anat. 2003; 202: 59-68
Stem cells to tissue: molecular, cellular and anatomical heterogeneity in skeletal muscle.
Curr. Opin. Genet. Dev. 2003; 13: 413-422
The behaviour of satellite cells in response to exercise: what have we learned from human studies?.
Pflugers Arch. 2005; 451: 319-327
Pax7 is required for the specification of myogenic satellite cells.
Cell. 2000; 102: 777-786
Transcriptional dominance of Pax7 in adult myogenesis is due to high-affinity recognition of homeodomain motifs.
Dev. Cell. 2012; 22: 1208-1220
The myogenic regulatory factors, determinants of muscle development, cell identity and regeneration.
Semin. Cell Dev. Biol. 2017; 72: 10-18
Expression of the muscle regulatory factor MRF4 during somite and skeletal myofiber development.
Dev. Biol. 1991; 147: 144-156
Myosin heavy chain isoforms regulate muscle function but not myofibril assembly.
EMBO J. 1996; 15: 4454-4459
The inflammatory response: friend or enemy for muscle injury?.
Br. J. Sports Med. 2003; 37: 284-286
Inflammatory cell response to acute muscle injury.
Med. Sci. Sports Exerc. 1995; 27: 1022-1032
Skeletal muscle injury—molecular changes in the collagen during healing.
Res. Exp. Med. 1985; 185: 95-106
Aberrant repair and fibrosis development in skeletal muscle.
Skelet. Muscle. 2011; 1: 1-20
Differentiation of muscle-derived cells into myofibroblasts in injured skeletal muscle.
Am. J. Pathol. 2002; 161: 895-907
Dystrophin protects the sarcolemma from stresses developed during muscle contraction.
Proc. Natl. Acad. Sci. U. S. A. 1993; 90: 3710-3714
Laminin polymerization induces a receptor-cytoskeleton network.
J. Cell Biol. 1999; 145: 619-631
Active peptides from the carboxyl-terminal globular domain of laminin α2 and Drosophila α chains.
FEBS Lett. 1996; 396: 37-42
Identification of α-dystroglycan binding sequences in the laminin α2 chain LG4-5 module.
Matrix Biol. 2010; 29: 143-151
Laminin enriched scaffolds for tissue engineering applications.
Adv. Tissue Eng. Regen. Med. Open Access. 2017; 2: 00033
Integrin α subunit ratios, cytoplasmic domains, and growth factor synergy regulate muscle proliferation and differentiation.
J. Cell Biol. 1996; 133: 169-184
The roles of RGD and grooved topography in the adhesion, morphology, and differentiation of C2C12 skeletal myoblasts.
Biotechnol. Bioeng. 2012; 109: 2104-2115
Increasing α7β1-integrin promotes muscle cell proliferation, adhesion, and resistance to apoptosis without changing gene expression.
Am. J. Physiol. Cell Physiol. 2008; 294: C627-C640
Screening of integrin-binding peptides in a laminin peptide library derived from the mouse laminin β chain short arm regions.
Arch. Biochem. Biophys. 2014; 550: 33-41
A synthetic peptide containing the IKVAV sequence from the A chain of laminin mediates cell attachment, migration, and neurite outgrowth.
J. Biol. Chem. 1989; 264: 16174-16182
Selection of peptides binding to the α5β1 integrin from phage display library.
J. Biol. Chem. 1993; 268: 20205-20210
Hepatocyte growth factor affects satellite cell activation and differentiation in regenerating skeletal muscle.
Am. J. Phys. Cell Phys. 2000; 278: C174-C181
In situ regeneration of skeletal muscle tissue through host cell recruitment.
Acta Biomater. 2014; 10: 4332-4339
Basic fibroblast growth factor promotes in vivo muscle regeneration in murine muscular dystrophy.
Neurosci. Lett. 1995; 202: 121-124
Regulation of skeletal muscle stem cells by fibroblast growth factors.
Dev. Dyn. 2017; 246: 359-367
Fibroblast growth factor and transforming growth factor beta repress transcription of the myogenic regulatory gene MyoD1.
Mol. Cell. Biol. 1989; 9: 3576-3579
Myostatin signals through Pax7 to regulate satellite cell self-renewal.
Exp. Cell Res. 2008; 314: 317-329
Vascular endothelial growth factor stimulates skeletal muscle regeneration in Vivo.
Mol. Ther. 2004; 10: 844-854
Effects of interleukin-6, leukemia inhibitory factor, and ciliary neurotrophic factor on the proliferation and differentiation of adult human myoblasts.
Cell. Mol. Neurobiol. 2008; 28: 113-124
Interleukin-6 is an essential regulator of satellite cell-mediated skeletal muscle hypertrophy.
Cell Metab. 2008; 7: 33-44
Interleukin-8 enhances myocilin expression, Akt-FoxO3 signaling and myogenic differentiation in rat skeletal muscle cells.
J. Cell. Physiol. 2019; 234: 19675-19690
Tumor necrosis factor-α inhibition of skeletal muscle regeneration is mediated by a caspase-dependent stem cell response.
Stem Cells. 2008; 26: 997-1008
IGF-1 induces skeletal myocyte hypertrophy through calcineurin in association with GATA-2 and NF-ATc1.
Nature. 1999; 400: 581-585
TNF inhibits notch-1 in skeletal muscle cells by Ezh2 and DNA methylation mediated repression: implications in Duchenne muscular dystrophy.
PLoS ONE. 2010; 5e12479
IL-6 improves myogenesis in long-term skeletal muscle atrophy via the JAK/STAT3 signalling pathway.
Int. J. Clin. Exp. Pathol. 2017; 10: 4023-4034
- SEO Powered Content & PR Distribution. Get Amplified Today.
- Platoblockchain. Web3 Metaverse Intelligence. Knowledge Amplified. Access Here.
- Source: https://www.cell.com/trends/biotechnology/fulltext/S0167-7799(22)00244-X?rss=yes
- 1
- 3d
- a
- access
- across
- Activation
- activity
- administration
- Adult
- After
- Aging
- aligned
- Alloy
- analysis
- and
- animal
- Application
- applications
- approaches
- ARM
- Assembly
- Association
- authors
- based
- basic
- beta
- between
- Biomaterials
- BONE
- Capacity
- carriers
- Cells
- central
- chain
- chains
- Changes
- changing
- Children
- Clinical
- clinical trials
- comparison
- complex
- considerations
- construct
- controlled
- Costs
- creation
- Culture
- delivery
- Derived
- Design
- Dev
- developed
- Development
- direct
- Display
- dna
- Dog
- domain
- domains
- Dominance
- drug
- during
- Early
- effect
- effects
- efficiency
- Electric
- embedded
- emerging
- enable
- energy
- Engineering
- enhanced
- enriched
- essential
- evaluation
- evidence
- Exercise
- exhibits
- explained
- factors
- fibers
- flow
- Focus
- following
- Force
- formation
- friend
- from
- function
- functional
- fusion
- generated
- Girl
- Growth
- heads
- hinders
- host
- HTTPS
- human
- Hybrid
- Identity
- Impact
- implications
- improve
- improves
- improving
- in
- integration
- isolated
- IT
- Leads
- learned
- levels
- Library
- List
- Long
- long-term
- loss
- maintains
- Mass
- materials
- mdx
- Media
- medicine
- Members
- Men
- methods
- mice
- migration
- model
- models
- module
- MOL
- molecular
- musculoskeletal
- network
- New
- normal
- obstacles
- ONE
- open
- organization
- Organized
- Overcome
- participation
- Patches
- phase
- phenomenon
- plato
- Plato Data Intelligence
- PlatoData
- potential
- present
- primary
- Production
- promise
- promote
- promotes
- RAT
- Rate
- recognition
- recovery
- recruitment
- reduces
- regenerative
- regions
- Regulate
- regulator
- regulatory
- repair
- Reports
- required
- research
- resembles
- Resistance
- response
- review
- Role
- roles
- safe
- satellite
- SCI
- Sequence
- Short
- signals
- similarities
- specification
- Sports
- stable
- Stem
- stem cells
- Still
- stimulates
- strategies
- strength
- studies
- Study
- Studying
- subsequent
- success
- Supports
- synergy
- synthetic
- system
- Technologies
- The
- their
- Therapeutic
- therapy
- three-dimensional
- Through
- tissues
- to
- today
- tool
- transforming
- treating
- treatment
- Trends
- trials
- Triple
- units
- Updates
- via
- viability
- vivo
- W
- What
- within
- without
- Women
- X
- young
- zephyrnet